Ownership and Moves in Rust
Contents
Two characteristics are desired from programming languages when it comes to managing memory:
- We’d like memory to be freed promptly, at a time of our choosing. This gives us control over the program’s memory consumption.
- We never want to use a pointer to an object after it’s been freed. This would be undefined behavior, leading to crashes and security holes.
These two characteristics seem to be mutually exclusive: freeing a value while pointers exist to it necessarily leaves those pointers dangling.
- 既想可以手动管理内存,又不想因为手动释放内存不当导致 dangling pointers 的出现。
Almost all major programming languages fall into one of two camps, depending on which of the two qualities they give up on:
- The “Safety First” camp uses garbage collection to manage memory, automatically freeing objects when all reachable pointers to them are gone.
- This eliminates dangling pointers by simply keeping the objects around until there are no pointers to them left to dangle. Almost all modern languages fall in this camp, from Python, JavaScript, and Ruby to Java, C#, and Haskell.
- Relying on garbage collection means relinquishing control over exactly when objects get freed to the collector. In general, garbage collectors are surprising beasts, and understanding why memory wasn’t freed when you expected can be a challenge.
- The “Control First” camp leaves you in charge of freeing memory.
- Your program’s memory consumption is entirely in your hands, but avoiding dangling pointers also becomes entirely your concern. C and C++ are the only mainstream languages in this camp.
Rust breaks the deadlock by restricting how your programs can use pointers. These restrictions brings just enough order to the chaos to allow Rust’s compile-time checks to verify that your program is free of memory safety errors: dangling pointers, double frees, using uninitialized memory, and so on. At run time, your pointers are simple addresses in memory, just as they would be in C and C++. The difference is that your code has been proven to use pointers safely.
These same rules also form the basis of Rust’s support for safe concurrent programming. Using Rust’s carefully designed threading primitives, the rules that ensure your code uses memory correctly also serve to prove that it is free of data races.
- A bug in a Rust program cannot cause one thread to corrupt another’s data, introducing hard-to-reproduce failures in unrelated parts of the system.
- The nondeterministic behavior inherent in multithreaded code is isolated to those features designed to handle it—mutexes, message channels, atomic values, and so on—rather than appearing in ordinary memory references.
Rust’s radical wager, the claim on which it stakes its success and that forms the root of the language, is that even with these restrictions in place, you’ll find the language more than flexible enough for almost every task and that the benefits—the elimination of broad classes of memory management and concurrency bugs—will justify the adaptations you’ll need to make to your style.
TL;DR
Moves and reference-counted pointers are two ways to relax the rigidity of the ownership tree. Borrowing references to values is a third way.
Ownership
In C or C++, an instance of some class owns some other object that it points to. This generally means that the owning object gets to decide when to free the owned object: when the owner is destroyed, it destroys its possessions along with it.
// c++
std::string s = "frayed knot";
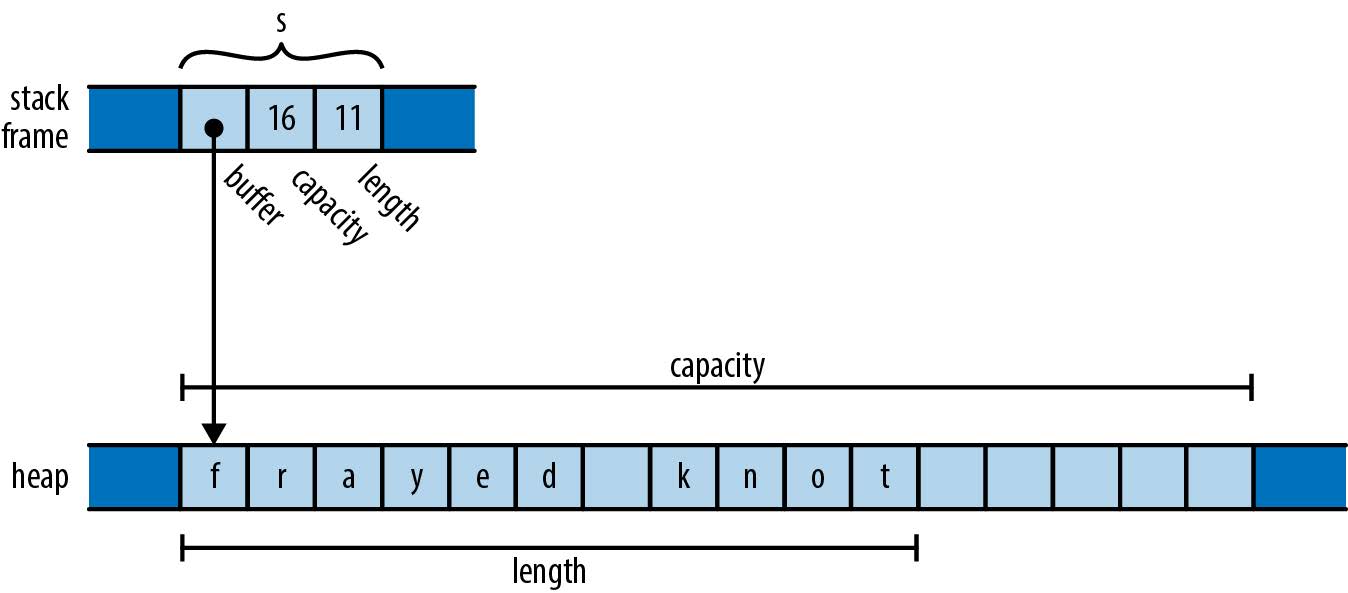
- The actual
std::string
object itself is always exactly three words long, comprising a pointer to a heap-allocated buffer, the buffer’s overall capacity (that is, how large the text can grow before the string must allocate a larger buffer to hold it), and the length of the text it holds now. These are fields private to thestd::string class
, not accessible to the string’s users. Astd::string
owns its buffer: when the program destroys the string, the string’s destructor frees the buffer.- In the past, some C++ libraries shared a single buffer among several
std::string
values, using a reference count to decide when the buffer should be freed. Newer versions of the C++ specification effectively preclude that representation; all modern C++ libraries use the approach shown here.
- In the past, some C++ libraries shared a single buffer among several
- In these situations it’s generally understood that although it’s fine for other code to create temporary pointers to the owned memory, it is that code’s responsibility to make sure its pointers are gone before the owner decides to destroy the owned object.
- You can create a pointer to a character living in a
std::string
’s buffer, but when the string is destroyed, your pointer becomes invalid, and it’s up to you to make sure you don’t use it anymore. The owner determines the lifetime of the owned, and everyone else must respect its decisions. - It’s just a convention that the standard library generally follows, and although the language encourages you to follow similar practices, how you design your own types is ultimately up to you.
- You can create a pointer to a character living in a
In Rust, the concept of ownership is built into the language itself and enforced by compile-time checks. Every value has a single owner that determines its lifetime. When the owner is freed—dropped, in Rust terminology—the owned value is dropped too.
- These rules are meant to make it easy for you to find any given value’s lifetime simply by inspecting the code, giving you the control over its lifetime that a systems language should provide.
A variable owns its value. When control leaves the block in which the variable is declared, the variable is dropped, so its value is dropped along with it.
fn print_padovan() {
let mut padovan = vec![1,1,1]; // heap buffer allocated here
for i in 3..10 {
let next = padovan[i-3] + padovan[i-2];
padovan.push(next);
}
println!("P(1..10) = {:?}", padovan);
} // heap buffer dropped here
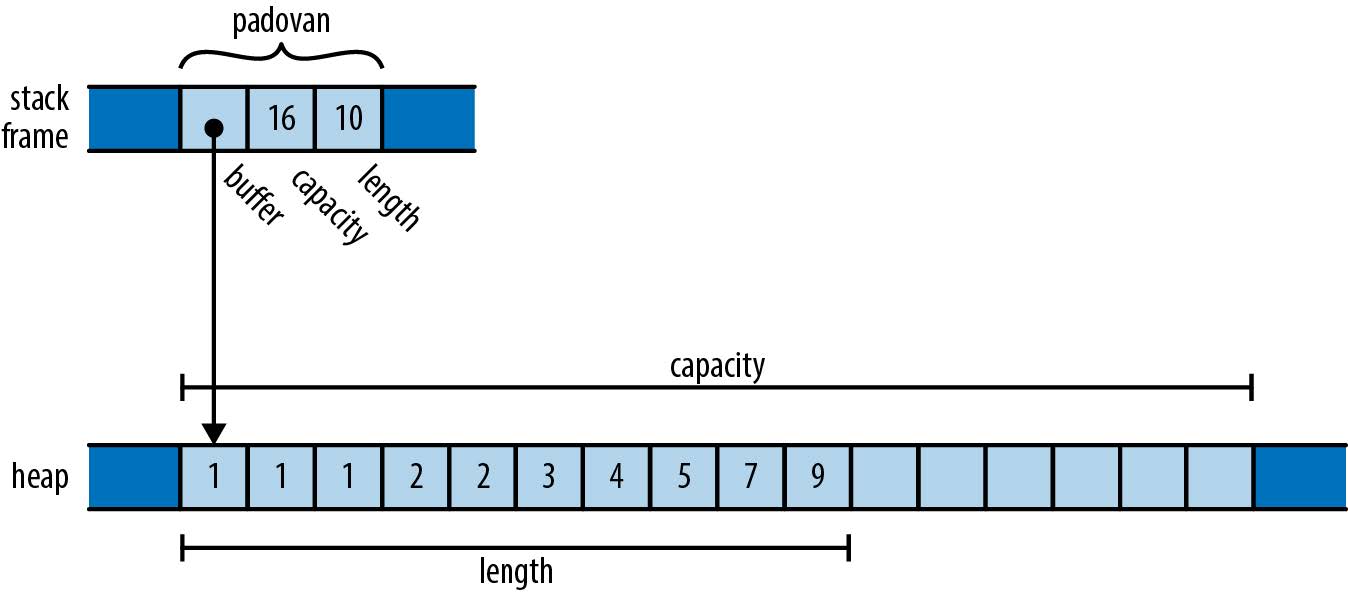
- The vector owns the buffer holding its elements. When the variable
padovan
goes out of scope at the end of the function, the program drops the vector. And since the vector owns its buffer, the buffer goes with it. - The words holding
padovan
’s pointer, capacity, and length live directly in the stack frame of theprint_padovan
function; only the vector’s buffer is allocated on the heap.
A Box<T>
is a pointer to a value of type T
stored on the heap. Calling Box::new(v)
allocates some heap space, moves the value v
into it, and returns a Box
pointing to the heap space. Since a Box
owns the space it points to, when the Box
is dropped, it frees the space too.
{
let point = Box::new((0.625, 0.5)); // point allocated here
let label = format!("{:?}", point); // label allocated here
assert_eq!(label, "(0.625, 0.5)");
} // both dropped here
-
When the program calls
Box::new
, it allocates space for a tuple of twof64
values on the heap, moves its argument(0.625, 0.5)
into that space, and returns a pointer to it. -
By the time control reaches the call to
assert_eq!
, the stack frame looks like this:- The stack frame itself holds the variables
point
andlabel
, each of which refers to a heap allocation that it owns. When they are dropped, the allocations they own are freed along with them.
- The stack frame itself holds the variables
Just as variables own their values, structs own their fields, and tuples, arrays, and vectors own their elements.
struct Person { name: String, birth: i32 }
let mut composers = Vec::new();
composers.push(Person { name: "Palestrina".to_string(),
birth: 1525 });
composers.push(Person { name: "Dowland".to_string(),
birth: 1563 });
composers.push(Person { name: "Lully".to_string(),
birth: 1632 });
for composer in &composers {
println!("{}, born {}", composer.name, composer.birth);
}
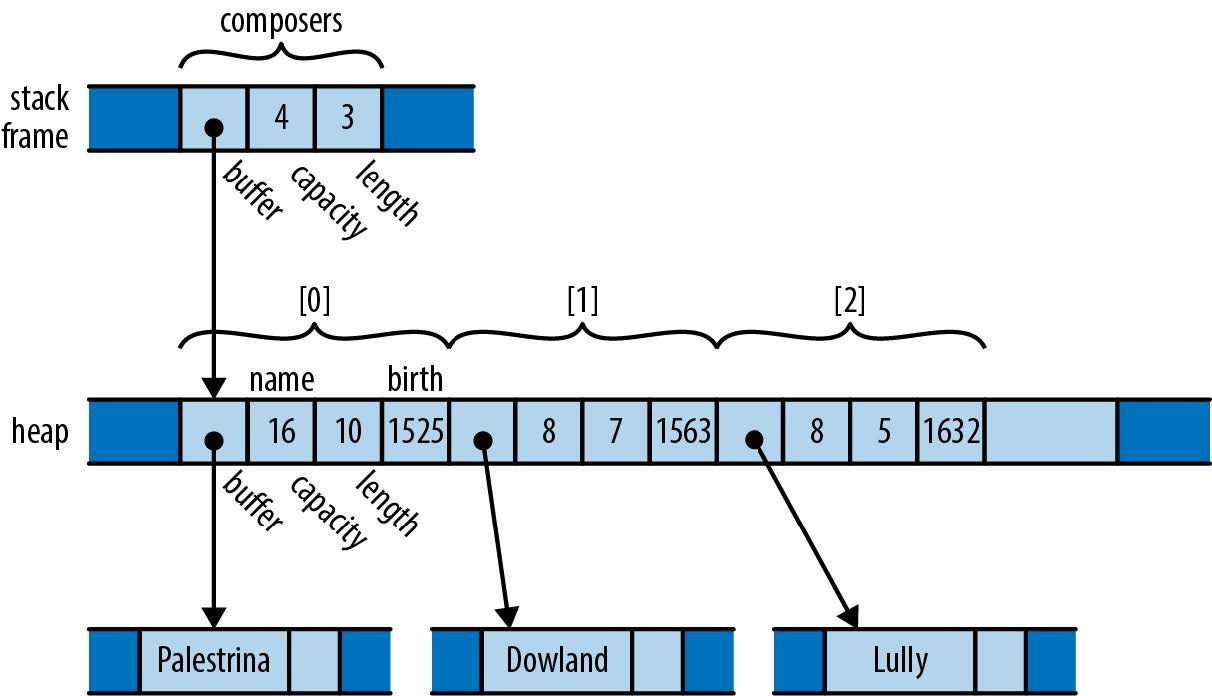
composers
owns a vector; the vector owns its elements, each of which is aPerson
structure; each structure owns its fields; and the string field owns its text.- When control leaves the scope in which
composers
is declared, the program drops its value and takes the entire arrangement with it.
Every value has a single owner, making it easy to decide when to drop it. But a single value may own many other values: for example, the vector composers
owns all of its elements.
The owners and their owned values form trees: your owner is your parent, and the values you own are your children. At the ultimate root of each tree is a variable; when that variable goes out of scope, the entire tree goes with it.
- It’s not a “tree” in the sense of a search tree data structure, or an HTML document made from DOM elements. Rather, we have a tree built from a mixture of types, with Rust’s single-owner rule forbidding any rejoining of structure that could make the arrangement more complex than a tree.
- Every value in a Rust program is a member of some tree, rooted in some variable.
Rust programs don’t usually explicitly drop values at all, in the way C and C++ programs would use free
and delete
. The way to drop a value in Rust is to remove it from the ownership tree somehow: by leaving the scope of a variable, or deleting an element from a vector, or something of that sort. At that point, Rust ensures the value is properly dropped, along with everything it owns.
In a certain sense, Rust is less powerful than other languages: every other practical programming language lets you build arbitrary graphs of objects that point to each other in whatever way you see fit. But it is exactly because Rust is less powerful that the analyses the language can carry out on your programs can be more powerful. Rust’s safety guarantees are possible exactly because the relationships it may encounter in your code are more tractable.
Rust’s “radical wager”: in practice, Rust claims, there is usually more than enough flexibility in how one goes about solving a problem to ensure that at least a few perfectly fine solutions fall within the restrictions the language imposes.
Rust extends the rigid concept of ownership in several ways to make it useful:
- You can move values from one owner to another. This allows you to build, rearrange, and tear down the tree. Very simple types like integers, floating-point numbers, and characters are excused from the ownership rules. These are called
Copy
types. - The standard library provides the reference-counted pointer types
Rc
andArc
, which allow values to have multiple owners, under some restrictions. - You can “borrow a reference” to a value; references are non-owning pointers, with limited lifetimes.
Each of these strategies contributes flexibility to the ownership model, while still upholding Rust’s promises.
Moves
In Rust, for most types, operations like assigning a value to a variable, passing it to a function, or returning it from a function don’t copy the value: they move it. The source relinquishes ownership of the value to the destination and becomes uninitialized; the destination now controls the value’s lifetime.
Rust programs build up and tear down complex structures one value at a time, one move at a time.
There’s actually significant variation from one school to another on how different languages have chosen to handle assignment.
# python
s = ['udon', 'ramen', 'soba']
t = s
u = s
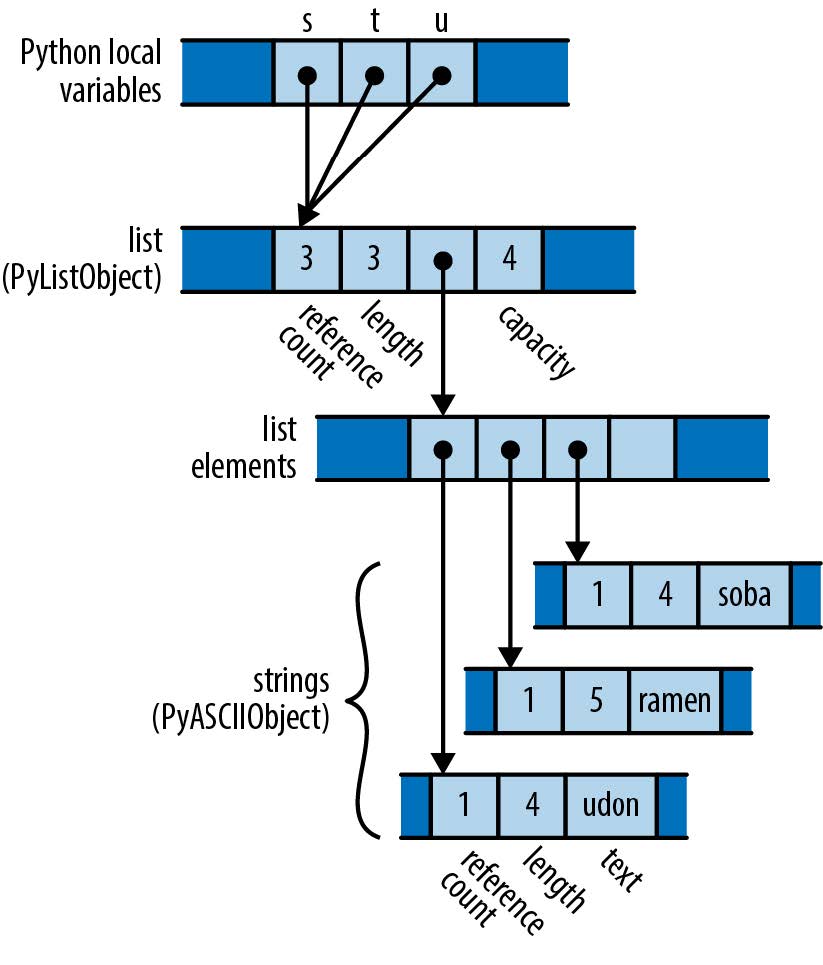
- Each Python object carries a reference count, tracking the number of values that are currently referring to it.
- Python implements assignment simply by making the destination point to the same object as the source, and incrementing the object’s reference count.
- Assignment in Python is cheap, but because it creates a new reference to the object, we must maintain reference counts to know when we can free the value.
// c++
using namespace std;
vector<string> s = { "udon", "ramen", "soba" };
vector<string> t = s;
vector<string> u = s;
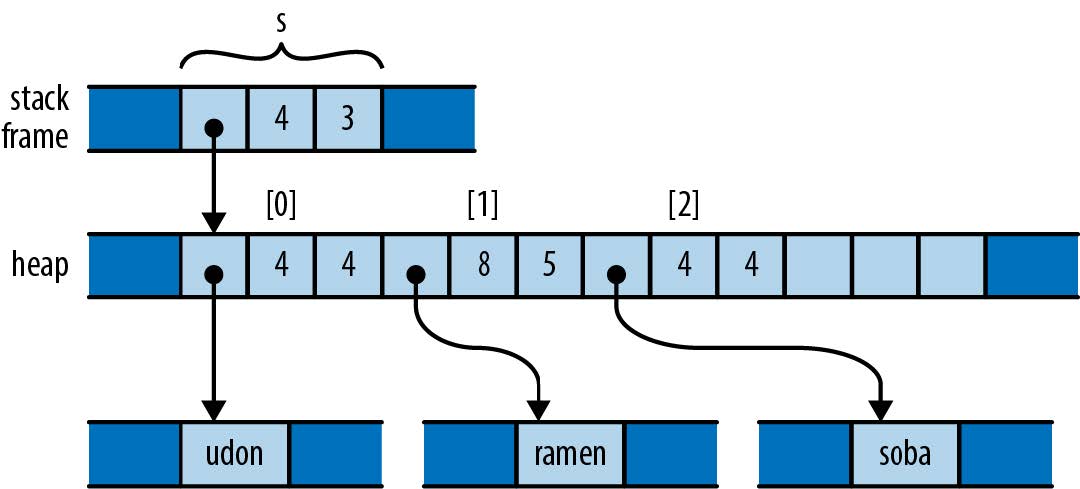
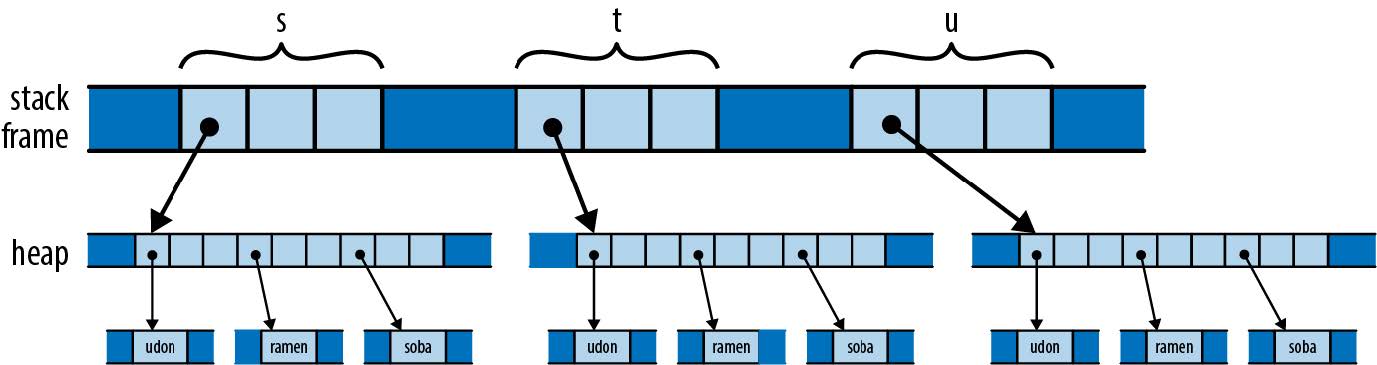
- Assigning a
std::vector
produces a copy of the vector in C++;std::string
behaves similarly. So by the time the program reaches the end of this code, it has actually allocated three vectors and nine strings- Depending on the values involved, assignment in C++ can consume unbounded amounts of memory and processor time.
- The advantage is that it’s easy for the program to decide when to free all this memory: when the variables go out of scope, everything allocated here gets cleaned up automatically.
In a sense, C++ and Python have chosen opposite trade-offs:
- Python makes assignment cheap, at the expense of requiring reference counting (and in the general case, garbage collection).
- C++ keeps the ownership of all the memory clear, at the expense of making assignment carry out a deep copy of the object.
- C++ programmers are often less than enthusiastic about this choice: deep copies can be expensive, and there are usually more practical alternatives.
// rust
let s = vec!["udon".to_string(), "ramen".to_string(), "soba".to_string()];
let t = s;
let u = s;
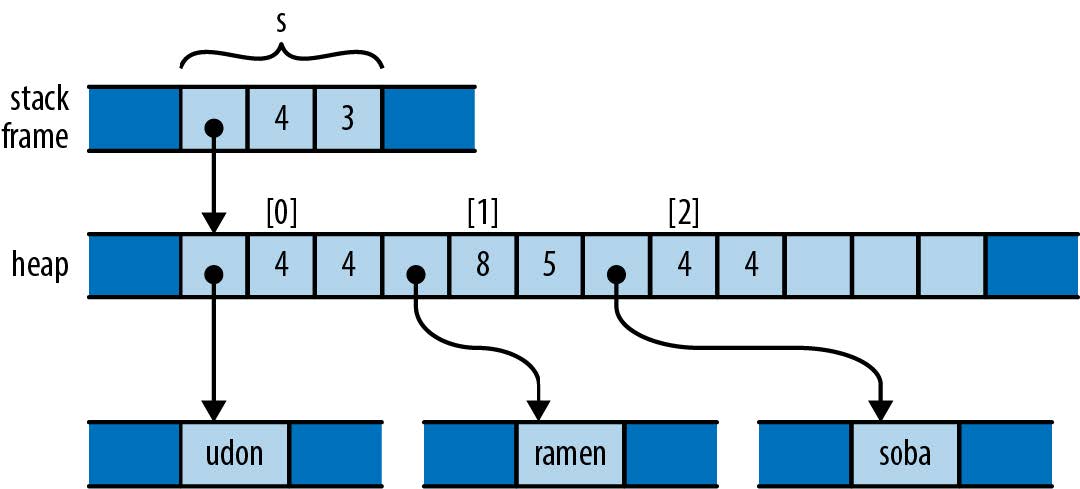
-
Like C and C++, Rust puts plain string literals like
"udon"
in read-only memory, so for a clearer comparison with the C++ and Python examples, we callto_string
here to get heap-allocatedString
values. -
The initialization
let t = s;
moved the vector’s three header fields froms
tot
; nowt
owns the vector.- The vector’s elements stayed just where they were, and nothing happened to the strings either.
- Every value still has a single owner, although one has changed hands.
- There were no reference counts to be adjusted.
- The compiler now considers
s
uninitialized.
-
let u = s;
would assign the uninitialized values
tou
. Rust prudently prohibits using uninitialized values, so the compiler rejects this code.
The consequences of Rust’s use of a move here:
-
Like Python, the assignment is cheap: the program simply moves the three-word header of the vector from one spot to another.
-
Like C++, ownership is always clear: the program doesn’t need reference counting or garbage collection to know when to free the vector elements and string contents.
-
You must explicitly ask for copies when you want them.
let s = vec!["udon".to_string(), "ramen".to_string(), "soba".to_string()]; let t = s.clone(); let u = s.clone();
- If you want to end up in the same state as the C++ program, with each variable holding an independent copy of the structure, you must call the vector’s
clone
method, which performs a deep copy of the vector and its elements. - You could also re-create Python’s behavior by using Rust’s reference-counted pointer types.
- If you want to end up in the same state as the C++ program, with each variable holding an independent copy of the structure, you must call the vector’s
More Operations That Move
If you move a value into a variable that was already initialized, Rust drops the variable’s prior value.
let mut s = "Govinda".to_string();
s = "Siddhartha".to_string(); // value "Govinda" dropped here
- When the program assigns the string
"Siddhartha"
tos
, its prior value"Govinda"
gets dropped first.
let mut s = "Govinda".to_string();
let t = s;
s = "Siddhartha".to_string(); // nothing is dropped here
t
has taken ownership of the original string froms
, so that by the time we assign tos
,s
is uninitialized. In this scenario, no string is dropped.
Rust applies move semantics to almost any use of a value. Passing arguments to functions moves ownership to the function’s parameters. Returning a value from a function moves ownership to the caller. Building a tuple moves the values into the tuple. And so on.
struct Person { name: String, birth: i32 }
let mut composers = Vec::new();
composers.push(Person { name: "Palestrina".to_string(),
birth: 1525 });
- Returning values from a function:
Vec::new()
constructs a new vector and returns, not a pointer to the vector, but the vector itself: its ownership moves fromVec::new
to the variablecomposers
.to_string
call returns a freshString
instance.
- Constructing new values:
- The
name
field of the newPerson
structure is initialized with the return value ofto_string
. The structure takes ownership of the string.
- The
- Passing values to a function:
- The entire
Person
structure, not a pointer to it, is passed to the vector’spush
method, which moves it onto the end of the structure. The vector takes ownership of thePerson
and thus becomes the indirect owner of the nameString
as well.
- The entire
Moving values around like this may sound inefficient, but there are two things to keep in mind.
- The moves always apply to the value proper, not the heap storage they own.
- For vectors and strings, the value proper is the three-word header alone; the potentially large element arrays and text buffers sit where they are in the heap.
- The Rust compiler’s code generation is good at “seeing through” all these moves; in practice, the machine code often stores the value directly where it belongs.
Moves and Control Flow
The general principle by which moves interact with more complicated code is that, if it’s possible for a variable to have had its value moved away and it hasn’t definitely been given a new value since, it’s considered uninitialized.
-
If a variable still has a value after evaluating an
if
expression’s condition, then we can use it in both branches.let x = vec![10, 20, 30]; if c { f(x); // ... ok to move from x here } else { g(x); // ... and ok to also move from x here } h(x); // bad: x is uninitialized here after either path uses it
-
Moving from a variable in a loop is forbidden unless we’ve definitely given it a new value by the next iteration.
let x = vec![10, 20, 30]; while f() { g(x); // bad: x would be moved in first iteration, uninitialized in second } let mut x = vec![10, 20, 30]; while f() { g(x); // move from x x = h(); // give x a fresh value } e(x);
Moves and Indexed Content
A move leaves its source uninitialized, as the destination takes ownership of the value. But not every kind of value owner is prepared to become uninitialized.
// Build a vector of the strings "101", "102", ... "105"
let mut v = Vec::new();
for i in 101 .. 106 {
v.push(i.to_string());
}
// Pull out random elements from the vector.
let third = v[2]; // error: Cannot move out of index of Vec
// | |
// | move occurs because value has type `String`, which does not implement the `Copy` trait
// | help: consider borrowing here: `&v[2]`
- Rust rejects the preceding code.
- For this to work, Rust would somehow need to remember that the third and fifth elements of the vector have become uninitialized, and track that information until the vector is dropped. In the most general case, vectors would need to carry around extra information with them to indicate which elements are live and which have become uninitialized. That is clearly not the right behavior for a systems programming language; a vector should be nothing but a vector.
- Rust suggests using a reference, in case you want to access the element without moving it. This is often what you want.
Three possibilities if you really do want to move an element out of a vector:
// Build a vector of the strings "101", "102", ... "105"
let mut v = Vec::new();
for i in 101 .. 106 {
v.push(i.to_string());
}
// 1. Pop a value off the end of the vector:
let fifth = v.pop().expect("vector empty!");
assert_eq!(fifth, "105");
// 2. Move a value out of a given index in the vector,
// and move the last element into its spot:
let second = v.swap_remove(1);
assert_eq!(second, "102");
// 3. Swap in another value for the one we're taking out:
let third = std::mem::replace(&mut v[2], "substitute".to_string());
assert_eq!(third, "103");
assert_eq!(v, vec!["101", "104", "substitute"]);
- Each one of these methods moves an element out of the vector, but does so in a way that leaves the vector in a state that is fully populated, if perhaps smaller.
Collection types like Vec
also generally offer methods to consume all their elements in a loop:
let v = vec!["liberté".to_string(),
"égalité".to_string(),
"fraternité".to_string()];
for mut s in v {
s.push('!');
println!("{}", s);
}
- When we pass the vector to the loop directly, as in
for ... in v
, this moves the vector out ofv
, leavingv
uninitialized. Thefor
loop’s internal machinery takes ownership of the vector and dissects it into its elements.- At each iteration, the loop moves another element to the variable
s
. - Since
s
now owns the string, we’re able to modify it in the loop body before printing it. - Since the vector itself is no longer visible to the code, nothing can observe it mid-loop in some partially emptied state.
- A “mid-loop” refers to the point at which the loop is currently executing, but has not yet reached its termination condition.
- At each iteration, the loop moves another element to the variable
If you do find yourself needing to move a value out of an owner that the compiler can’t (and shouldn’t) track, you might consider changing the owner’s type to something that can dynamically track whether it has a value or not.
struct Person { name: Option<String>, birth: i32 }
let mut composers = Vec::new();
composers.push(Person { name: Some("Palestrina".to_string()),
birth: 1525 });
// let first_name = composers[0].name; // error “cannot move out of index
// | |
// | move occurs because value has type `Option<String>`, which does not implement the `Copy` trait
// | help: consider borrowing here: `&composers[0].name`
let first_name = std::mem::replace(&mut composers[0].name, None);
assert_eq!(first_name, Some("Palestrina".to_string()));
assert_eq!(composers[0].name, None);
// same effect
let first_name = composers[0].name.take();
- The
replace
call moves out the value ofcomposers[0].name
, leavingNone
in its place, and passes ownership of the original value to its caller. - Using
Option
this way is common enough that the type provides atake
method for this very purpose.
Copy Types: The Exception to Moves
So far the values being moved involve vectors, strings, and other types that could potentially use a lot of memory and be expensive to copy. Moves keep ownership of such types clear and assignment cheap. For simpler types like integers or characters, this sort of careful handling really isn’t necessary.
let string1 = "somnambulance".to_string();
let string2 = string1;
let num1: i32 = 36;
let num2 = num1;
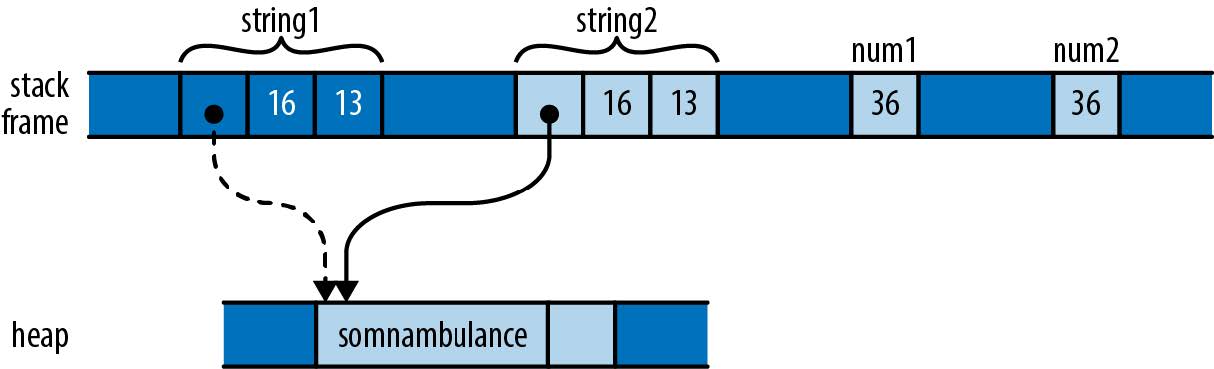
- Assignment moves
string1
tostring2
so that we don’t end up with two strings responsible for freeing the same buffer. - Assignment
num1
tonum2
makes a completely independent copy ofnum1
.- An
i32
is simply a pattern of bits in memory; it doesn’t own any heap resources or really depend on anything other than the bytes it comprises. By the time we’ve moved its bits tonum2
, we’ve made a completely independent copy ofnum1
.
- An
- Whereas it serves an essential purpose to treat
string1
as valueless, treatingnum1
that way is pointless; no harm could result from continuing to use it. The advantages of a move don’t apply here, and it’s inconvenient.
Assigning a value of a Copy
type copies the value, rather than moving it. The source of the assignment remains initialized and usable, with the same value it had before.
- Passing
Copy
types to functions and constructors behaves similarly.
The standard Copy
types include all the machine integer and floating-point numeric types, the char
and bool
types, and a few others. A tuple or fixed-size array of Copy
types is itself a Copy
type.
Only types for which a simple bit-for-bit copy suffices can be Copy
.
String
is not aCopy
type, because it owns a heap-allocated buffer.Box<T>
is notCopy
because it owns its heap-allocated referent.- The
File
type, representing an operating system file handle, is notCopy
; duplicating such a value would entail asking the operating system for another file handle. - The
MutexGuard
type, representing a locked mutex, isn’tCopy
: this type isn’t meaningful to copy at all, as only one thread may hold a mutex at a time.
As a rule of thumb, any type that needs to do something special when a value is dropped cannot be Copy
: a Vec
needs to free its elements, a File
needs to close its file handle, a MutexGuard
needs to unlock its mutex, and so on. Bit-for-bit duplication of such types would leave it unclear which value was now responsible for the original’s resources.
By default, user-defined types like struct
and enum
types are not Copy
.
#[derive(Copy, Clone)]
struct Label { number: u32 }
fn print(l: Label) { println!("STAMP: {}", l.number); }
let l = Label { number: 3 };
print(l);
println!("My label number is: {}", l.number);
// | let l = Label { number: 3 };
// | - move occurs because `l` has type `Label`, which does not implement the `Copy` trait
// | print(l);
// | - value moved here
// | println!("My label number is: {}", l.number);
// | ^^^^^^^^ value borrowed here after move
- User-defined types being non-Copy is only the default. If all the fields of your struct are themselves
Copy
, then you can make the typeCopy
as well by placing the attribute#[derive(Copy, Clone)]
above the definition,
Whether a type is Copy
or not has a big effect on how code is allowed to use it. Copy
types are more flexible, since assignment and related operations don’t leave the original uninitialized. But for a type’s implementer, the opposite is true: Copy
types are very limited in which types they can contain, whereas non-Copy
types can use heap allocation and own other sorts of resources. So making a type Copy
represents a serious commitment on the part of the implementer: if it’s necessary to change it to non-Copy
later, much of the code that uses it will probably need to be adapted. That’t why eligible user-defined types aren’t automatically Copy
types.
While C++ lets you overload assignment operators and define specialized copy and move constructors, Rust doesn’t permit this sort of customization.
In Rust, every move is a byte-for-byte, shallow copy that leaves the source uninitialized. Copies are the same, except that the source remains initialized.
This does mean that C++ classes can provide convenient interfaces that Rust types cannot, where ordinary-looking code implicitly adjusts reference counts, puts off expensive copies for later, or uses other sophisticated implementation tricks. But the effect of this flexibility on C++ as a language is to make basic operations like assignment, passing parameters, and returning values from functions less predictable.
One of Rust’s principles is that costs should be apparent to the programmer. Basic operations must remain simple. Potentially expensive operations should be explicit, like the calls to clone
that make deep copies of vectors and the strings they contain.
Rc and Arc: Shared Ownership
Although most values have unique owners in typical Rust code, in some cases it’s difficult to find every value a single owner that has the lifetime you need; you’d like the value to simply live until everyone’s done using it.
For these cases, Rust provides the reference-counted pointer types Rc
and Arc
. These are entirely safe to use: you cannot forget to adjust the reference count, create other pointers to the referent that Rust doesn’t notice, or stumble over any of the other sorts of problems that accompany reference-counted pointer types in C++.
The Rc
and Arc
types are very similar; The only difference between them is that an Arc
is safe to share between threads directly—the name Arc
is short for atomic reference count—whereas a plain Rc
uses faster non-thread-safe code to update its reference count. If you don’t need to share the pointers between threads, there’s no reason to pay the performance penalty of an Arc
, so you should use Rc
; Rust will prevent you from accidentally passing one across a thread boundary. The two types are otherwise equivalent.
use std::rc::Rc;
// Rust can infer all these types; written out for clarity
let s: Rc<String> = Rc::new("shirataki".to_string());
let t: Rc<String> = s.clone();
let u: Rc<String> = s.clone();
assert!(s.contains("shira"));
assert_eq!(t.find("taki"), Some(5));
println!("{} are quite chewy, almost bouncy, but lack flavor", u);
s.push_str(" noodles"); // error: cannot borrow data in an `Rc` as mutable
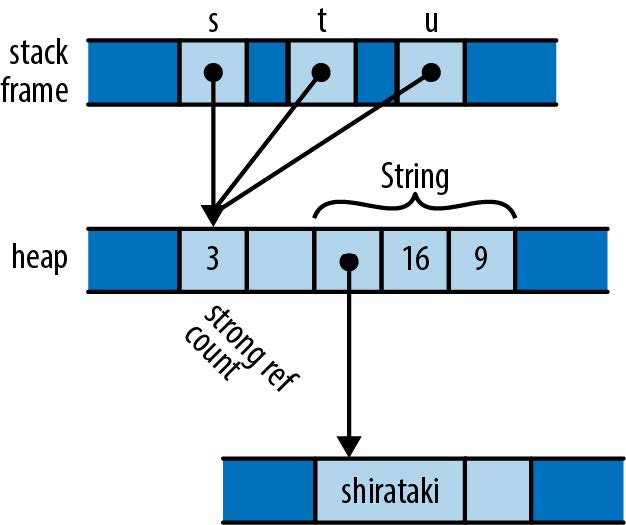
- For any type
T
, anRc<T>
value is a pointer to a heap-allocatedT
that has had a reference count affixed to it. - Cloning an
Rc<T>
value does not copy theT
; instead, it simply creates another pointer to it and increments the reference count. - Each of the three
Rc<String>
pointers is referring to the same block of memory, which holds a reference count and space for theString
. - The usual ownership rules apply to the
Rc
pointers themselves, and when the last extantRc
is dropped, Rust drops theString
as well. - You can use any of
String
’s usual methods directly on anRc<String>
. - A value owned by an
Rc
pointer is immutable. Rust’s memory and thread-safety guarantees depend on ensuring that no value is ever simultaneously shared and mutable. Rust assumes the referent of anRc
pointer might in general be shared, so it must not be mutable.
One well-known problem with using reference counts to manage memory is that, if there are ever two reference-counted values that point to each other, each will hold the other’s reference count above zero, so the values will never be freed.
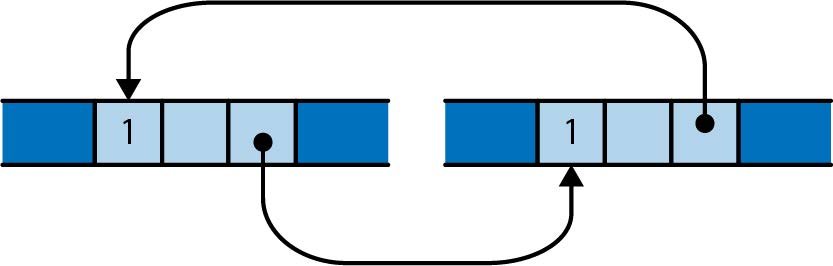
- It is possible to leak values in Rust this way, but such situations are rare. You cannot create a cycle without, at some point, making an older value point to a newer value. This obviously requires the older value to be mutable. Since
Rc
pointers hold their referents immutable, it’s not normally possible to create a cycle. - Rust does provide ways to create mutable portions of otherwise immutable values; this is called interior mutability. If you combine those techniques with
Rc
pointers, you can create a cycle and leak memory. - You can sometimes avoid creating cycles of
Rc
pointers by using weak pointers, std::rc::Weak .
References